Additional Projects
Additional Research Projects
Project Goal & Background
Sodium-ion batteries are the most technologically advanced of the “Beyond Lithium-Ion” chemistries. The high abundance and low cost of sodium-containing precursors and the similarity to lithium-ion analogs make these devices particularly attractive for grid storage and vehicle-to-grid applications, which are particularly cost- and resource-sensitive.
Because graphite does not intercalate sodium to an appreciable extent, hard carbon anodes are used in commercial sodium-ion batteries, but there are safety concerns due to the very low potentials at which sodium ions insert. We are currently investigating sustainable alternatives to hard carbon anodes, based on abundant, cheap titanium oxides.
Of particular interest are stepped layered titanates such as those with lepidocrocite structures. The electrochemical properties of these materials can be manipulated through judicious substitutions, inclusion of vacancies, and heat treatments. For example, as shown in the figure below, a vacancy-containing structure (NTO) delivers significantly greater capacity than one with partial lithium substitution.
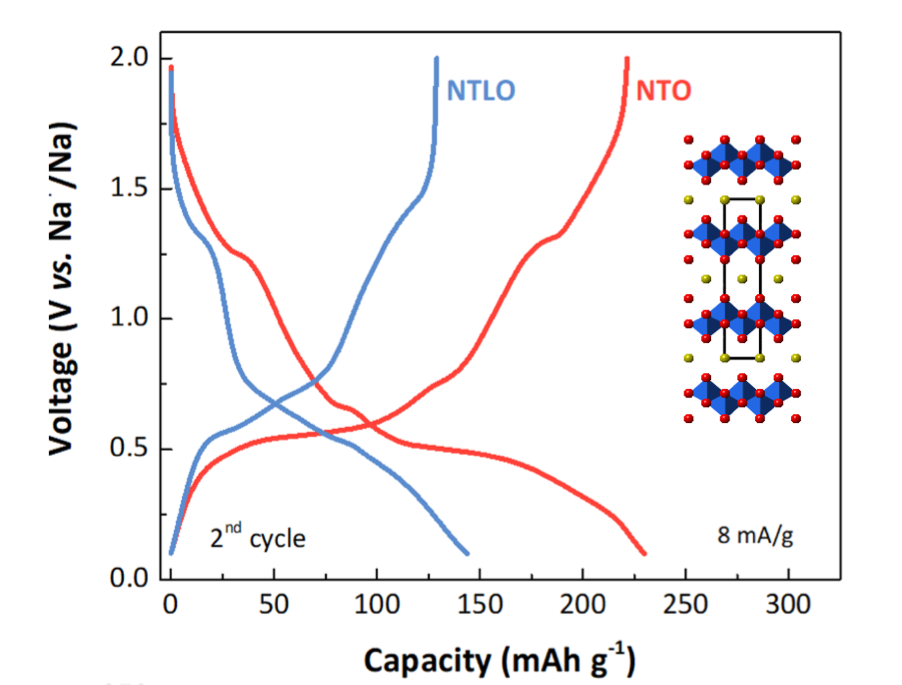
Project Goal & Background
The goal of this project is to develop and use our experimental capabilities to develop deeper understanding of the steps in electrode manufacturing, and to make this information available to assist researchers and manufacturers in both tuning processes for existing products and commercializing next-generation electrode components.
Electrode processing involves several steps, which each involve a large number of process decisions, whether made implicitly or explicitly. For instance, the “coating” or “casting” step in battery manufacturing involves putting a layer of a slurry onto a foil current collector.
This is affected by all of the choices that influenced the slurry properties at the time of casting (component selection and amounts, mixing order, mixing schedule, environment, etc.), in addition to all of the decisions in choosing and operating the coating equipment (slurry treatment following mixing, and for doctor blade casting: blade design, blade height, casting speed, environment, etc.).
Furthermore, electrode fabrication processes at the laboratory scale can be substantially different than at the manufacturing scale, which complicates the scale-up of new battery components into commercial products.
Tasks for FY22
- Conduct hard X-ray microradiography observations of drying coatings at the Advanced Light Source (ALS) user facility at Berkeley Lab.
- Refine design of our custom automated miniature coating / drying line robot used in the radiography studies to improve fidelity to conditions at the manufacturing scale, and repeat Step 1.
- Use our observations to develop models of electrode manufacturing processes.
- Release our controller package as open source software.
Recent Highlights
We have recently completed two sessions at the ALS beamline 8.3.2 with our miniature automated coating / drying line robot. We successfully recorded image sequences of drying coatings in profile, and observed surprising behavior, which we are documenting in a manuscript to be submitted for review shortly.
Project Goal & Background
To enable lithium battery cathodes fully based on earth-abundant (EA) elements, fundamental understanding of key parameters that control a material’s properties and performances, as well as the corrections among them at the particle-level, is essential. This can be difficult to achieve on conventional aggregated secondary particles, as isolating specific parameters of interest from less controlled ones, such as porosity, grain boundaries, particle size and size distribution, is often challenging if not impossible.
Researchers at Berkeley Lab are developing uniform, well-formed single-crystal model systems as a platform to investigate the complex role of dopants/substituents and how they may be used to improve the performance of layered, lithium transition-metal oxide cathodes with a minimal amount of Co and/or Ni.
Combined with electrochemical analysis, the synthesis and characterization based effort aims to gather a fundamental understanding of how EA dopant/substitution impacts key processes such as oxygen redox and oxygen loss, transition-metal migration and dissolution, and surface reconstruction on cathode materials. A fundamental understanding is also needed of how EA dopant/substitution impacts the corrections between the processes and electrochemical performance metrics, such as hysteresis, impedance, rate capability and cycling stability. This knowledge will help with the design and development of next-generation EA cathodes that have improved performance, including single-crystal based EA cathode materials.
Project Goal & Background
As the world transitions to relying increasingly on renewable energy sources such as wind and solar, there is a critical need for large-scale energy storage to provide energy to the grid when the wind isn’t blowing or the sun isn’t shining. Batteries are widely considered an attractive solution to this challenge as they have fast response times and are easily scalable. In order to compete with fossil fuels, the combined cost of renewables and storage must be lower than the current cost of power generation.
To achieve this, we have designed a battery system that both utilizes low-cost materials and a design, which makes the recycling process easier, further lowering costs. We have assembled a prototype system with flow-through electrodes, an aqueous (non-flammable) electrolyte, and earth-abundant materials. Our projected chemical cost of $54/kWh is lower than those of both lithium ion and vanadium redox flow batteries.
Tasks for FY22
Task 1: Develop a working prototype with ultra-thick flow through electrodes. We have assembled a preliminary working prototype with 1cm thick electrodes, up to 85% coulombic efficiency, and 55 cycles.
Task 2: Explore additional chemistries that will lower the costs further. We have identified a Mn-Zn formulation that would lower the chemical cost to $42/kWh.
Task 3: Assess cycle life and address any failure mechanisms. We are validating methods to address dendrite growth at the zinc anodes to prolong system lifetime.
Project Goal & Background
The demand for lithium is soaring as it is a crucial ingredient in green technologies, but the current methods of production are slow, expensive, wasteful, and damaging to the environment. We are quantifying current lithium processing emissions and waste generation and highlight six priorities for the lithium industry to overcome these challenges and meet global sustainability targets:
- Extract lithium in fewer steps
- Convert waste into valuable commodities
- Process minerals electrochemically underground
- Make electrodes out of raw ores
- Expand mining alongside recycling globally
- Coordinate policies, boost research and communicate
Research Overview & Tasks
Lithium-ion batteries demonstrate excessive charge transfer resistance at the cathode/electrolyte interface at low temperatures. Berkeley Lab investigated the effect of partially replacing ethylene carbonate with gamma-butyrolactone on low temperature performance.
Additive A1 at a composition of 0.5% and 0.75% shows the best overall performance at low-temperature. (From Battaglia Lab).
Towards this effort, Berkeley Lab discovered an additive that reduces the low temperature charge transfer resistance by a factor of three with negligible impact on the rest of the cell’s performance at nominal and high temperatures.